Parts and Functions of the Cardiovascular System (Circulatory System)
In Lecturio . The purpose of the blood circulation system of the human body is the convective transport of respiratory gases, nutrients, hormones, and warmth. The blood acts as a vehicle that is pumped by the heart through the blood vessels and back to the heart. The blood circulation is a rapidly adjustable system that is vitally important for maintaining the body functions. High blood pressure (arterial hypertension) is one of the most common diseases in our society. Thus, a physician must know and understand the blood circulation. This article provides an extensive overview.
Table of Contents
- Structure of the Blood Circulation
- Fluid Mechanics of the Blood Circulation
- Arterial Hemodynamics
- Windkessel Function of the Aorta
- Low-Pressure System
- Microcirculation
- Blood Pressure Regulation
- Pathophysiology of Blood Circulation
- Fetal Blood Circulation
- Popular Exam Question on Blood Circulation
- References
Are you more of a visual learner? Check out our online video lectures and start your vascular medicine course now for free!
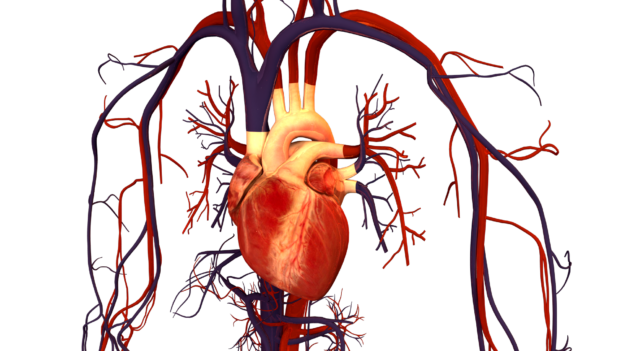
Image: “Human Heart and Circulatory System.” by Bryan Brandenburg. License: CC BY-SA 3.0
Structure of the Blood Circulation
The blood circulation of the human body consists of pipes that are connected in series and in parallel. These are the blood vessels which form a closed circulatory system. In the center, there are two pumps: the two heart ventricles (Latin: ventriculi cordis). They maintain the pressure gradient which creates blood flow.The left ventricle pumps oxygenated blood through the aorta into the systemic or greater circulation. Here, it spreads into large arteries that lead to the organs. These arteries are arranged parallel to each other.
The large arteries branch further into smaller arteries until they become arterioles. These, in turn, branch into capillaries where substances are exchanged through diffusion. The number of the vessels constantly increases while their diameter decreases.
Out of the capillaries, the blood flows into the smaller veins via venules. The veins drain blood into the superior and inferior vena cava by means of the muscle pump and the venous valves (which prevent the blood from flowing back into the periphery).
Both superior and inferior vena cavae lead to the right atrium of the heart (Latin: atrium cordis dextrum). In the venous system, the number of vessels constantly decreases while their diameter increases. With atrial contraction, the blood is pumped into the right ventricle.
The right ventricle ejects the blood via the pulmonary trunk into the pulmonary circulation. Here, it spreads into the pulmonary arteries, which further branch out within the lung until they finally become arterioles and capillaries. Now, the blood can be oxygenated by means of diffusion across the alveolar membranes while carbon dioxide diffuses out.
Via venules, veins, and the four large pulmonary veins, the blood is led back to the heart and into the left atrium (Latin: atrium cordis sinistrum). With atrial contraction, the oxygenated blood flows into the left ventricle and has thus completed one round through the circulation. One round normally takes 60 seconds. This circulation time can be determined with a dye.
Fluid Mechanics of the Blood Circulation
Basically, the blood vessels form a pipe system through which the blood passes. Thus, it is necessary to take a look at the physical laws of fluids.I is the flow of a volume element per period of time:
I = ∆V / ∆t (in m3/s)
The flow velocity vm – averaged over the cross section – is the covered distance of the blood corpuscles per period of time:
vm = ∆I / ∆t (in m/s)
If the cross section of the vessel remains constant, the volume element ∆v is the product of cross section and covered distance:
∆v = ∆I * Q (in m3)
This results in the condition of continuity: At constant flow, velocity decreases with increasing cross section.
I = (∆I / ∆t) * ∆Q = vm * Q or vm = I / Q
The cross section is taken as the sum of all parallel vessels. Thus, the cross section of the capillary system is 500 times the cross section of the aorta. Hence, the flow velocity of the blood decreases further and further from aorta (0.25 m/s) to the capillaries (0.0005 m/s).If a fluid flows through a pipe system, friction occurs both between the elements of the fluid and the wall of the pipe. This dynamic tenacity is described by viscosity η. It results from the quotient of shear stress (τ = force/area) and the velocity gradient (γ = dv/dx):
η = τ / γ (in Pa s)
If all fluid layers and, hence, the fluid particles that move parallel to the wall of the vessel, this is referred to as laminar flow. Since the friction at the wall of the vessel is greatest, a parabolic velocity profile results.Under these circumstances, the Law of Hagen-Poiseuille applies:
I = (r4 π∆p) / (8ηl) with ∆p = pressure difference, r = inner radius of the vessel, and l = length of the vessel
If one considers Ohm’s law I = ∆p / R, the following results for the resistance R:
R = (8 η l) / r4 π
Flow velocity is thus proportional or, vice-versa, inversely proportional to the fourth power of the inner radius of the vessel.Not every flow is laminar. If the critical Reynolds number (≥ 2000) is exceeded, the flow becomes turbulent, and the model of layers does not apply anymore. Turbulent flow creates vortexes and thus leaves the parabolic velocity profile of the laminar flow. In cases of stenosis or anemia, an increase in flow velocity in the vessels can result in turbulent flow. Unlike laminar flow, turbulent flow can be heard. This occurs with a venous hum, carotid bruits, cardiac murmurs and is the base of the technique of blood pressure measurement.
Arterial Hemodynamics
When the left ventricle contracts, the stroke volume is ejected into the aorta. The blood is accelerated and hence the pressure in the part of the aorta closest to the heart rises. The increase in pressure creates a stretch in the elastic wall of the aorta. Here, an increase in cross section can be measured.Windkessel Function of the Aorta
This mechanism of the aorta that stores a part of the volume in the increased cross section is referred to as the Windkessel function of the aorta. The Windkessel function is responsible for the fact that the increase in pressure during systole in the blood circulation system is lower than it would be in a rigid pipe system. If the pressure in the aorta decreases, i.e. during diastole, the aortic wall is elastic and recoils, pushing the stored blood volume forward. A continuous blood flow results.Pressure Pulse, Flow Pulse, and Cross Section or Volume Pulse
The rhythmic ejection of blood out of the heart into the vessels leads to pulse waves. Here, one has to distinguish between pressure pulse, flow pulse, and cross section or volume pulse. While pressure and volume pulse spread with almost the same velocity, the flow pulse is significantly slower. With 0.2 m/s, the low velocity is distinctly below the pulse wave velocity of 5 m/s.
In more distal sections of the aorta, the flow pulse is characterized by an early diastolic phase of reverse flow and an adjoining forward flow. The phase of reverse flow occurs as early as in the aortic trunk. If the pressure in the aorta exceeds the one in the left ventricle, a fraction of the blood flows back to the heart. This brings on the closure of the aortic valve and completes systole. With a temporal delay, this reverse flow continues in the peripheral vessels with the reverse flow amplitude decreasing more and more. Even in the small arteries, the by now diastolic phase of reverse flow can be easily measured.
If you plot the pressure in arterial vessel with the time, the pressure pulse graph looks like this:
In the aorta, a vessel close to the heart, a dicrotic notch can be seen, ( see above figure) which is caused by the reverse flow for the closure of the aortic valve. This marks the end of systole. In the arteries further away from the heart, this dicrotic notch cannot be found anymore. Instead, a double peak is present. It is caused by the reflection of the waves.
Note: Two cars of the same weight drive towards each other with the same speed and collide head-on. The immediate impact pressure is high. Also wave pressures add together. After the impact, both cars stand. They have stopped each other. Just like this, the wave currents cancel each other out.
Pulse Wave Velocity
With increasing distance to the heart, the pulse wave velocity also increases. This happens because of two reasons: Firstly, the ratio between wall thickness and inner radius decreases. Secondly, the wall composition of the smaller arteries and arterioles changes. The elastic fibers of the adventitia decrease, while the muscular part of the media expands. This leads to an increase in rigidity or tensile strength of the peripheral arteries and, thus, to an increase of the pulse wave velocity overall.
PWV = √(Eh / 2rp) with E = tensile strenght, h = wall thickness, r = inner radius, and p = pressure
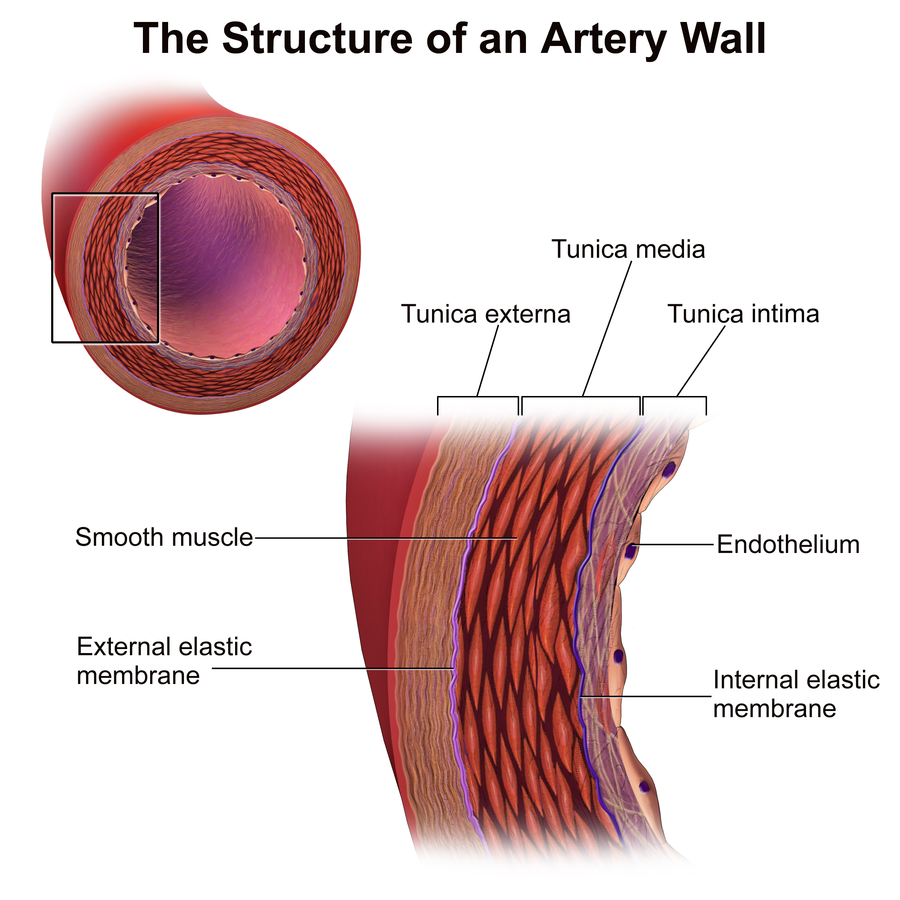
Image: “Artery Wall Structure” by Bruce Blaus. License: CC BY 3.0
In older people, the tensile strenght of the arteries and with it the pulse wave velocity increases. Other than age and distance from the heart, the mean arterial pressure has an influence on the pulse wave velocity, as the equation states.
The mean arterial blood pressure corresponds to the mean value of the pressure over the period of one pulse. The lowest pulse pressure at the end of the diastole is the diastolic blood pressure (Pdias). Normally, it is around 80 mmHg. The highest pressure reached in systole is the systolic blood pressure (Psys). Its regular value is 120 mmHg.
It can be calculated by using the following equation:
MAP= Pdias + 1/3 (Psys – Pdias)
In large blood vessels, the blood pressure decreases only slightly. Only when the so-called resistance vessels are reached, it significantly drops. The terminal arteries and arterioles are such resistance vessels. With vasoconstriction or vasodilation, i.e. the narrowing and widening of their radius, they can influence the peripheral flow resistance.
The flow resistance of all vessels of the greater circulation is the total peripheral resistance. With the cardiac output, it has an influence on the mean arterial pressure:
MAP = CO * TPR
Low-Pressure System
The low-pressure system includes all veins (vessels leading to the heart) of the greater circulation, the right heart, the lung vessels, and the left atrium. During diastole, the left ventricle is also part of the low-pressure system.In contrast to the high-pressure system of the arteries, the maximum blood pressure in the low-pressure system amounts to 20 mmHg.
The mean pressure in the large veins close to the heart is referred to as central venous pressure. It roughly equals the pressure in the right atrium, which is 0-2 cmH2O.
Due to the low-pressure amplitude, the venous blood transport has to occur in a different manner than in the arterial system. Here, the most important pump is not the heart but the muscles. For the flow to the heart to be ensured, the peripheral veins are situated inside the muscle compartments. At muscle contraction, the pressure in the vein rises. The venous valves guarantee that the blood is only transported towards the heart. They let the blood pass in only one direction, and that is towards the heart.
When the body rests, the pressure in the veins is influenced by cardiac activity. The venous pulse is characterized by the a-wave, the c-wave, the minimum (x), the v-wave, and the y-descent.
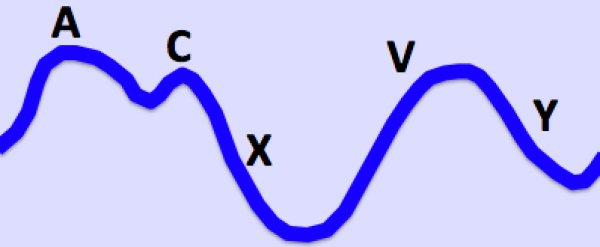
Image: “Jugular Venous Pulse” by Ecgtocardiology. License: CC BY-SA 3.0
With 15-20 mmHg, the peripheral veins have the highest pressure in the low-pressure system. It decreases in laying position and with increasing proximity to the heart. While standing, the hydrostatic pressure has to be considered. Thus, the pressure in the foot veins is highest, while in the head veins it is even lower than on heart level.
The greatest physiological pressure drop can be measured where the inferior vena cava passes through the diaphragm. This is a result of the higher current resistance that is caused by the narrow passing point. The point of equilibrium is five to ten centimeters below the diaphragm. Here, the pressure is independent from the position of the patient’s body. In the chest the negative pleural pressure plays a significant role in aiding blood return to the heart.
Due to its significantly higher compliance (ability to distend), the low-pressure system contains up to 85 % of the whole blood volume. For this reason, veins are referred to as capacitance vessels. When blood loss occurs, the stored (slow moving blood) blood can be quickly mobilized via sympathetic venoconstriction. It is moved to the general circulation in order to maintain the blood pressure.
When quickly changing from a lying to a standing position, the so-called orthostasis takes place. Gravity would push blood volume into the veins of the lower extremities. Thus, venous return, central venous pressure, stroke volume and hence systolic blood pressure would decrease upon standing. In order to counteract this imbalance, both total peripheral resistance and heart rate increase. With the muscle pump, the shifted blood can be transported back to the heart.
Microcirculation
The term microcirculation encompasses arterioles, capillaries, and post-capillary venules. Also, the terminal lymphatic vessels are included.Substance Exchange
In the terminal vascular bed, that is the capillary network, the blood circulation performs its main function: the exchange of substances between blood and interstitium.Lipid-soluble substances like oxygen and carbon dioxide can diffuse through the plasma membranes of the single-layer capillaries.
Fick’s law of diffusion states that the number of parts that diffuse per period of time depends on the area that is available for diffusion.In this case, the whole endothelial area is available. The transportation rate of the lipid soluble substances is not limited by the diffusion speed but only by capillary circulation. If it decreases, so does the substance exchange. This is also referred to as circulation limited exchange.
Water soluble substances and water itself need porins in the cell membrane to be able to pass the endothelium. The capillary water exchange amounts to circa 55 L/min, whereas substance exchange is nearly balanced, meaning that an equal amount of molecules diffuses from the blood into the interstitium and vice-versa.
Substance exchange only occurs if pressure and concentration gradients force the particles to pass through the endothelium. In this case, the effective filtration pressure determines the fluid transport through the capillary wall:
Peff = ∆p – ∆π = (pc – pis) – (πc – πis)
with p=hydrostatic pressure and π = colloid osmotic pressureIf one wants to determine the filtrated volume per period of time Jv, the filtration coefficient Kf (=hydraulic conductivity x area) has to be considered. This results in Starling’s equation.
Jv = Kf * peff
At filtration, Jv is greater than zero; at reabsorption, it is smaller than zero.In the arterial side of the terminal vascular bed, in the capillaries, the transmural pressure ∆p is higher than the difference of the oncotic pressures ∆π. This means that at this point, water and dissolved substances can flow from the blood into the interstitium. On the way to the venous side, the hydrostatic pressure drops and a filtration equilibrium is established. At the venous side of the capillaries, the transmural pressure gradient decreases, resulting in an effective reabsorption, as the pressure is directed from the outside to the inside of the capillary. Fluid from the tissue flows back into the blood vessels.
All in all, a little more fluid is filtrated than reabsorbed. The fluid remaining in the tissue is removed by the blind-ended lymphatic vessels. They lead water further up the lymphatic system and finally through the thoracic duct into the left venous arch, i.e. back into the blood circulation.
Lymphatic vessels are afferent, efferent and lymphatic ducts.
Blood Pressure Regulation
Remember the following important formula:
MAP = CO * TPR or MAP = SV * HF * TPR
with SV = stroke volume and HF = heart rateOne or more of these factors have to be manipulated in order to regulate blood pressure. To do this this, the body uses central and local mechanisms.
Central Blood Pressure Regulation
Sensors located in the carotid sinus (on both sides), the aortic arch, and the brachiocephalic trunk detect wall tension. These sensors trigger the baroreceptor reflex.When blood pressure increases, the action potential frequency in the pressoreceptors increases. The information is processed in the circulatory center in the rostroventrolateral medulla oblongata. Here, it causes a vagal stimulation. Its negative chronotropic effect on the heart decreases heart frequency and, therefore, the blood pressure. Also, the peripheral resistance vessels dilate in order to decrease total peripheral resistance.
When blood pressure is low, the action potential frequency in the pressoreceptors decreases. Via the circulatory center, this inhibits the vagal activity so that the sympathetic nervous system has a greater effect. Its positive chronotropic and inotropic effects lead to an increase in heart frequency and stroke volume. Overall, cardiac output is increased. Also, peripheral vessels contract to increase total peripheral resistance. This way, the blood pressure is brought to its original level.
The pressoreceptors therefore stabilize blood pressure. Without them, measured blood pressure values vary significantly in amplitude. However, the sensors can adjust to new ranges. This way, they do not necessarily inhibit too high a blood pressure, they only ensure that it remains relatively constant.
Hormones and vasoactive peptides also contribute to the regulation of blood pressure. Catecholamines play a very important role in this. Adrenaline and noradrenaline concentrations increase to the tenfold level with physical strain. Via the α1-receptors, they mediate vasoconstriction, which results in increased mean arterial pressure. However, adrenaline also has affinity to β-receptors. In physiological concentrations, this affinity dominates and adrenaline leads to vasodilation in the skeletal muscles, the liver, and the myocardium.
Angiotensin II leads to an increase in blood pressure. It plays an important role in the blood vessels of the kidney and only indirectly contributes to the systemic blood pressure.
Adiuretin or antidiuretic hormone, ADH, induces the opening of aquaporin channels in the dells lining the collecting tubules of the kidney. Thus more water is reabsorbed and less is excreted. This is considered an indirect regulation of the blood pressure. Additionally, in the event of a severe blood loss, adiuretin makes all vessels constrict, except for the cerebral and coronary vessels which react with vasodilation. This way, adiuretin ensures sufficient blood supply of the heart and brain whenever a great blood loss occurs.
Atrial natriuretic peptide (ANP) or atriopeptin is released when the atrial distension increases. This is either caused by too much blood volume or an increased central venous pressure. ANP leads to vasodilation, preferentially in the venous system. This redistributes blood volume from central to peripheral regions. Additionally, the ANP-mediated dilation of the kidney vessels results in diuresis. As a result, blood volume decreases.
Local Blood Pressure Regulation
Locally, blood pressure is mainly regulated by the autoregulation of the blood vessels and thus only affects total peripheral resistance.The Bayliss effect describes the mechanism by which vessels keep organ blood supply constant, even when arterial blood pressure is fluctuating. For this, mechano-sensitive cation channels are situated in the wall of the vessel. They open as soon as they register increased blood pressure. This makes Ca2+-ions flow into the smooth muscles, where they bind with calmodulin. This complex activates the myosin light-chain kinase, which in turn activates myosin via phosphorylation. Myosin can now interact with actin and reactive vasoconstriction takes place. It prevents the organ from being supplied with too much blood at high arterial blood pressure.
In addition, endothelial cells can register increased shear tension τ and produce more nitrogen monoxide (NO) in response. NO causes vasodilation, which counteracts high blood pressure.
Pathophysiology of Blood Circulation
Arterial Hypertension
In industrial countries, arterial hypertension is a widespread disease. This is not very surprising if one considers the so-called risk factors (factors that increase the risk for the disease) like smoking, obesity, stress, inactivity, and extensive salt and alcohol consumption.Arterial hypertension is diagnosed when systolic blood pressure is constantly above 140 mmHg or when diastolic blood pressure is above 90 mmHg. In more than 80% of the cases, this is a primary or essential hypertension. It does not have any apparent cause. In contrast to this, secondary hypertension is usually the consequence of another disease, like kidney disease or an endocrine disorder.
Symptoms of arterial hypertension are headaches, dizziness, vision impairments, and nausea. For treatment, beta-blockers and ACE-inhibitors are the most common medication. Beta-blockers block the β-receptors and, thus, the effect of noradrenaline on the heart. This way, heart rate is decreased and with it, the blood pressure. ACE-inhibitors, in turn, competitively inhibit the angiotensin converting enzyme, which transforms angiotensin I to angiotensin II. The concentration of angiotensin II drops and with it the blood pressure.
Arteriosclerosis
Arteriosclerosis is the narrowing of blood vessels due to accumulation of plaques and other depositions. Risk factors for this disease are old age, male gender, but also inactivity, smoking, obesity, high cholesterol, and high blood pressure. In its initial stages, arteriosclerosis does not show any symptoms, but it can lead to other diseases like myocardial infarction or stroke, the destruction of myocardial or brain tissue due to impairment of blood circulation. Treatment consists of a change in nutrition, more activity, coagulation inhibitors, surgical vessel widening, or bypasses.Varicosities/ Varicose Veins
Varicosities form due to an innate weakness of connective tissue. They are enlarged veins, which are most commonly noticed at the lower limbs. During longer periods of sitting or standing, the blood is not sufficiently pumped towards the heart by the muscle pump and it accumulates in the legs. This results in stretching of the veins.If their wall structure is disturbed, the stretching can be so extensive that the venous valves cannot close anymore. Due to gravity, the blood flow is reversed and is not directed toward the heart anymore. Normally, the blood can drain via anastomoses between deep and superficial veins of the leg. The superficial veins are abnormally stretched and become tortuous. In milder cases, compression stockings and cold compresses are an effective treatment. If varicosities are advanced, surgical elimination of the affected veins can be considered.
Thrombosis
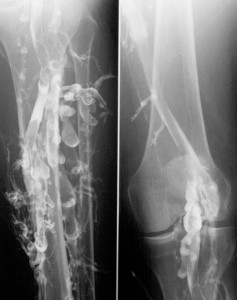
Image: “Phlebographie bei tiefer Beinvenenthrombose” by Hellerhoff. License: CC BY-SA 3.0
A blood clot can form in a vessel and occlude it or detach and migrate (in which case it is called embolus), and then obstruct a distal vessel. Venous thrombosis more frequently results in lung embolism, while arterial thrombosis normally leads to heart or brain infarction. In order to prevent thrombosis, coagulation inhibitors are prescribed and immobile patients have to wear compression stockings .
Shock
Shock is defined as a mismatch of oxygen supply and demand. It is classified according to its causes. Hypovolemic shock is caused by too little blood volume. Cardiogenic shock occurs due to insufficient pump performance of the heart. Septic shock is caused by pathogens in the blood and anaphylactic shock is brought on by an allergic reaction.Shock symptoms include a drop in blood pressure, cold sweat, pale skin, and sensation of thirst, mental confusion. The shock has to be treated immediately and appropriately. A dose of fresh plasma or iso-osmolar infusions are treatment options for hypovolemic shock. Also, bleeding must be stopped. Cardiogenic shock is counteracted by elevation of the torso. Additionally, the performance of the heart should be increased with medications and, if the left heart is failing and water accumulates in the lungs making breathing difficult artificial respiration should be initiated. Furthermore, the exact cause for shock should be diagnosed and treated as rapidly as possible.
Nearly 70% of patients with septic shock die. Transfusion of blood products and artificial respiration are supposed to keep the cardiovascular parameters in the physiological range. Histamine antagonists, adrenaline, and prednisolone are used in the treatment of anaphylactic shock.
Fetal Blood Circulation
The fetal blood circulation has to function without lung circulation since the fetal lung is not inflated and thus blood cannot be oxygenated. The oxygen-rich blood is provided by the maternal placenta and flows through the umbilical vein. It has a branch to the portal vein, which continues in the ductus venous that leads directly to the inferior vena cava. This way, the portal system is skipped.Oxygen-rich blood reaches the right atrium and skips the lung circulation via two physiological shunts. Either it can access the left atrium directly from the right atrium through the foramen ovale cordis or it can flow through the ductus arteriosus. The latter connects the pulmonary trunk with the aorta. With these two right-to-left shunts, the oxygenated blood accesses the left atrium or the greater circulation.
After substance transport, the arterial, deoxygenated blood flows through the umbilical arteries, branches of the internal iliac (or hypogastric) arteries, to reach the placenta where it is again oxygenated by diffusion of oxygen from the maternal circulation into the placental chorionic villi.
After birth, the lung inflates and thus, the lung circulation opens. This way, a change in pressures occurs, which results in the closure of the right-to-left shunts. The umbilical vessels obliterate. The ductus venosus also regresses and remains as the round ligament of the liver in the adult human.
Popular Exam Question on Blood Circulation
The solutions can be found below the references.1. The mean arterial blood pressure is defined as
- The sum of systolic and diastolic blood pressure.
- The arterial blood pressure in the middle of the ejection phase.
- The arterial blood pressure averaged over a pulse cycle.
- The value in the middle of the systolic and the diastolic blood pressure.
- The difference between the second power of the systolic and the second power of the diastolic blood pressure.
- 10 %
- 25 %
- 30 %
- 40 %
- 45 %
- PWV decreases with increasing distance to the heart.
- PWV decreases with decreasing tensile modulus.
- PWV decreases with increasing age.
- PWV decreases with increasing blood pressure.
- PWV decreases with increasing wall-thickness/radius relation.
Comentários
Enviar um comentário